This research uses a case study of a prototypical higher education campus renovation project to investigate and model a “smart” energy retrofit—one that considers the carbon payback as well as the cost payback of the renovation to target strategic energy retrofit measures that provide maximum carbon reductions with minimum carbon and cost investment.
The study tested an innovative process that incorporated several interrelated analytical methodologies to determine the optimal building renovation scope for maximum carbon reductions. These included thermal analysis to quantify the thermal resistance of individual components of the envelope, energy modeling to calibrate and determine whole building performance, and life cycle assessment to calculate embodied impacts. Using these tools in concert with cost estimating allowed the design team and owner to evaluate the financial and environmental return on investment of potential interventions in the existing building envelope, building systems, and primary energy sources.
This case study demonstrates a replicable process to optimize both embodied and operational carbon through iterative analysis. The process illustrates that not all energy conserving measures are worth pursuing when taken in the context of life cycle carbon and costs—a deep energy retrofit is not necessarily a smart energy retrofit. Additionally, energy retrofits should consider solutions that are appropriate to make immediate reductions while enabling further reductions through the future availability of greener energy sources. To reduce emissions from the building sector and achieve critical climate targets, the design and construction industry must rigorously analyze tradeoffs of embodied versus operations impacts, rather than defaulting to traditional best practice assumptions to meet critical climate targets.
Context
To meet the goals of the Paris Climate Agreement, we must reduce global greenhouse gas emissions by at least 65% in the next decade. It is imperative to seek carbon reduction strategies that reduce emissions in the near term, not over the next 50 years or over the life of a building; this is the concept of the time value of carbon. Buildings play a key role as they represent 39% of global emissions—roughly 28% from building operations and 11% from materials and construction. Building reuse therefore represents a critical two-part strategy to meeting reduction targets: it reduces embodied carbon emissions by reusing resource-intensive building components, avoiding the need for production of new materials; and it provides opportunities for dramatic reductions in operational emissions through improvements to energy efficiency. This strategy has the added benefit of greatly reducing upfront carbon emissions, making it a particularly effective strategy to reduce emissions in the critical near-term. However, reuse strategies are not all equally effective. While the architecture, engineering, and construction (AEC) industry has long assumed that deep energy retrofits are the most sustainable way to reuse a building, this view neglects the high embodied carbon cost of energy retrofit measures. Without understanding total carbon emissions and when they occur over a building’s life, renovations miss the opportunity to maximize critical near-term carbon reductions. The case study presented here examines a prototypical higher education campus renovation project to investigate what a “smart” energy retrofit looks like-one that considers the carbon payback as well as the cost payback of the renovation to target strategic energy retrofit measures that provide maximum carbon reductions with minimum carbon and cost investment.
Smart Energy Retrofit
Sustainable building reuse and decarbonization of the existing built environment have traditionally focused on driving down operational energy use by employing all available strategies, an approach termed “deep energy retrofit.” According the Rocky Mountain Institute, a deep energy retrofit is “…a whole building analysis and construction process that achieves much larger energy cost savings—sometimes more than 50% reduction—than those of simpler energy retrofits.” The New Buildings Institute defines a deep energy retrofit as “…a retrofit project that achieves at least 30% energy savings in a building.” Regardless of the exact threshold, these definitions account for only operational energy use. This approach is fundamentally problematic in that the upfront carbon emissions associated with deep energy retrofits create a carbon debt, which emissions savings from improved efficiency may not pay back for decades, exceeding our global carbon reduction deadlines.
Given the urgent global need to reduce carbon in the near term, the goal of the case study presented here was to define a “smart” energy retrofit: one which results in total carbon savings—including accounting for total life cycle emissions over the next ten years. This smart retrofit would strategically improve building energy performance through minimal addition of new material, select retrofit measures that had the greatest carbon impact with the least financial investment and disruption to the building, and position the building within the context of the greening grid and utility upgrades to maximize carbon reductions over time.
Case Study Introduction
This energy retrofit study was commissioned by a college to evaluate the optimal approach to retrofitting two mid-century residence halls. The study was conducted by Goody Clancy (project lead for architecture and life cycle assessment), Thornton Tomasetti (building analytics and envelope consultant), and van Zelm Engineers (MEP consultant). The existing buildings presented typical issues for structures of that era, including the need for envelope repairs, systems at the end of life, and accessibility challenges. The building envelope itself also presented common challenges to energy efficiency, including little existing insulation and significant thermal bridges, or areas in the exterior envelope that allow increased heat transfer. The college had also recently established an ambitious carbon neutrality target. This study provided the opportunity to develop a retrofit approach for these specific buildings and to evaluate how routine renewals of existing buildings could support progress toward their recently defined carbon neutrality target, producing a model for future renewals on the campus. With these goals in mind, the team agreed on the following objectives:
- Establish method for designing a “smart” energy retrofit project
- Understand which retrofit approaches have the best financial and carbon return on investment
- Draw conclusions about energy retrofit approaches that are applicable to future campus renewal projects
- Create a project that supports the campus’ carbon neutrality goals within budgetary constraints.
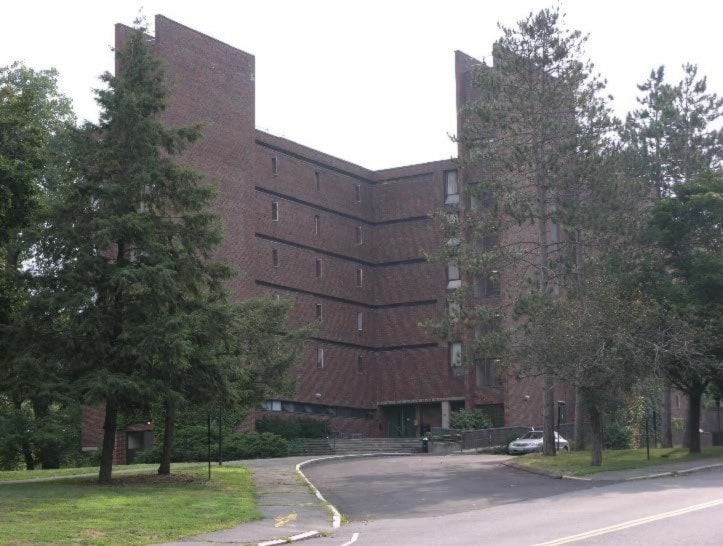
The study process followed a process that included goal-setting to understanding of the college’s priorities and project constraints, an intensive existing conditions assessment to ascertain both the physical condition of the buildings as well as their thermal and energy performance, two stages of analysis to assess a broad range of options and then understand how to compose the into an optimal project, and, finally, a discussion of scope building and phasing to support planning that balanced the immediate need for renewal with mid-term utility planning and space use goals.
Scope Evaluation Categories
To assess the feasibility of a deep energy retrofit, an extensive list of envelope and systems energy conservation measures were evaluated for their impacts on annual energy performance. In total, the team studied 125 individual scope items, including 50 energy conservation measures (ECMs). The measures fell into four categories:
Exterior envelope end-of-life replacements
- Window replacement: Options with a range of U-value, SHGC, and infiltration improvements
- Roof insulation: Insulation options ranging from R-30 (code baseline) to R-60 (super-insulation)
Exterior envelope performance upgrades
- Interior Insulation: Options ranging from 1” to 6” at all physically feasible locations as well as at targeted locations
- Exterior insulation: Insulation options ranging from inventions at significant thermal bridges to full exterior overclad
Building-level HVAC upgrades
- Options ranging from reducing the hot water temperatures supplied to existing radiators, to replacing the existing radiators with Runtal-style radiators, to providing both heating and cooling to each occupant room through radiant ceiling panels or VRFs. All including adequate ventilation for indoor air quality purposes. Where technically possible, options explored heating only (the existing condition) and the addition of cooling.
Primary energy source changes
- Options including connecting to the campus chilled water line for additional cooling, installing condensing boilers on site, depending on a geothermal source to meet all heating and cooling needs, and installing solar PV and solar thermal panels.
Some measures, such as window replacement and heating equipment upgrades, aligned with deferred maintenance priorities, while others, like adding exterior insulation, were solely targeted towards reducing the building’s operational energy consumption. While some measures were unlikely to be implemented due to cost or aesthetic impact, they were included in the study and assessed in order to document the impacts of these measures and assist the College in planning for future renovation projects.
Scope Evaluation Criteria
Each scope item was evaluated across a series of criteria including primary and secondary scope drivers (e.g., deferred maintenance status, energy efficiency, programmatic enhancements), future maintenance implications, thermal comfort impacts, user controllability, applicability to other buildings, deferred maintenance status, annual operational carbon reduction, and embodied carbon impacts. For the purposes of calculating total carbon impact, the last two metrics are defined as follows:
- Annual Operational Carbon Reduction: the reduction in carbon emissions resulting from annual building operations relative to the existing condition
- Embodied Carbon: upfront carbon emissions across life cycle stages from resource extraction to end-of-life of the materials associated with each item
For this study, the annual operation carbon reduction was evaluated for all ECMs and the embodied carbon impact was calculated for all exterior envelope end-of-life replacements and upgrades. For scope items that were modeled for both embodied and operational carbon impacts, the team quantified the combined carbon impact in relation to time using carbon payback period:
- Carbon Payback Period: the amount of time in years it takes for operational carbon savings to recover the embodied carbon expended at the time of construction
Scope Evaluation Process
The study began with an existing conditions assessment to observe and document the existing buildings. This assessment focused on existing envelope and systems components that would be impacted by energy retrofit interventions, including the identification of components in need of replacement. The existing conditions assessment consisted of a visual examination of the building envelope and systems, including thermal imaging to validate envelope thermal performance, exterior masonry and roof probes, and blower door testing at typical rooms to quantify air leakage through existing windows. Material samples were also collected to enable future testing for material properties needed as inputs to hygrothermal (WUFI) and other analyses that may be required in subsequent design phases.
The first round of analytical modeling was conducted to identify opportunities for greatest impact on energy efficiency, including thermal, energy, and comfort modeling. The architectural team conducted thermal modeling in THERM to quantify heat transfer at existing thermal bridges, test options for various insulation configurations, and establish the optimal R-value targets for different components. The consultant team completed the first round of full building energy modeling, using Open Studio both to calibrate the energy model of the existing conditions as well as to analyze 50 individual ECMs, understand the relative impacts of each intervention, and quickly establish the highest possible operational energy and carbon reductions. Due to the high number variables in flux during this round of analysis, the findings were most useful to compare relative impacts of different target values for one type of measure-for example double versus triple glazing or new windows versus insulation, rather than to select specific measures that would be optimal for the project’s carbon savings.
While the first round of analysis evaluated each measure individually, the second round of analytical modeling evaluated the interrelationships of ECMs and environmental impacts and included parametric energy modeling and life cycle assessment. The parametric energy modeling, using Open Studio, analyzed 240 combinations of ECMs to identify particular bundles that optimized energy use, carbon emissions, and cost reduction. Life cycle assessment, or embodied carbon analysis, conducted with Tally, quantified the embodied carbon emissions of envelope options and relative savings of substituting biogenic materials to store carbon, specifically for insulation and finish materials.
For the second round of modeling, a parametric approach was essential to accurately compare the potential savings for envelope measures, building-level systems upgrades, and options for primary source energy upgrades in combination. During this round of modeling, the team combined individual scope items into likely scope bundles, capturing the interrelationships of retrofit approaches and refining the projected operational savings of each scope item beyond the initial round of energy modeling. Once the calibrated operational carbon savings were established for individual scope items based on parametric model iterations that assumed likely scope combinations, the envelope scope items could be evaluated for operational carbon savings relative to the embodied carbon impact of the measure’s materials. The operational and embodied impacts were combined to identify the carbon payback period of individual scope items.
The final step of the evaluation process, establishing the scope priorities for current and future work, focused on identifying scope items necessary to facilitate future upgrades, items that provided the most cost-effective path towards significant energy savings, and combinations of items that would enable future savings as campus-wide energy sources become more efficient.
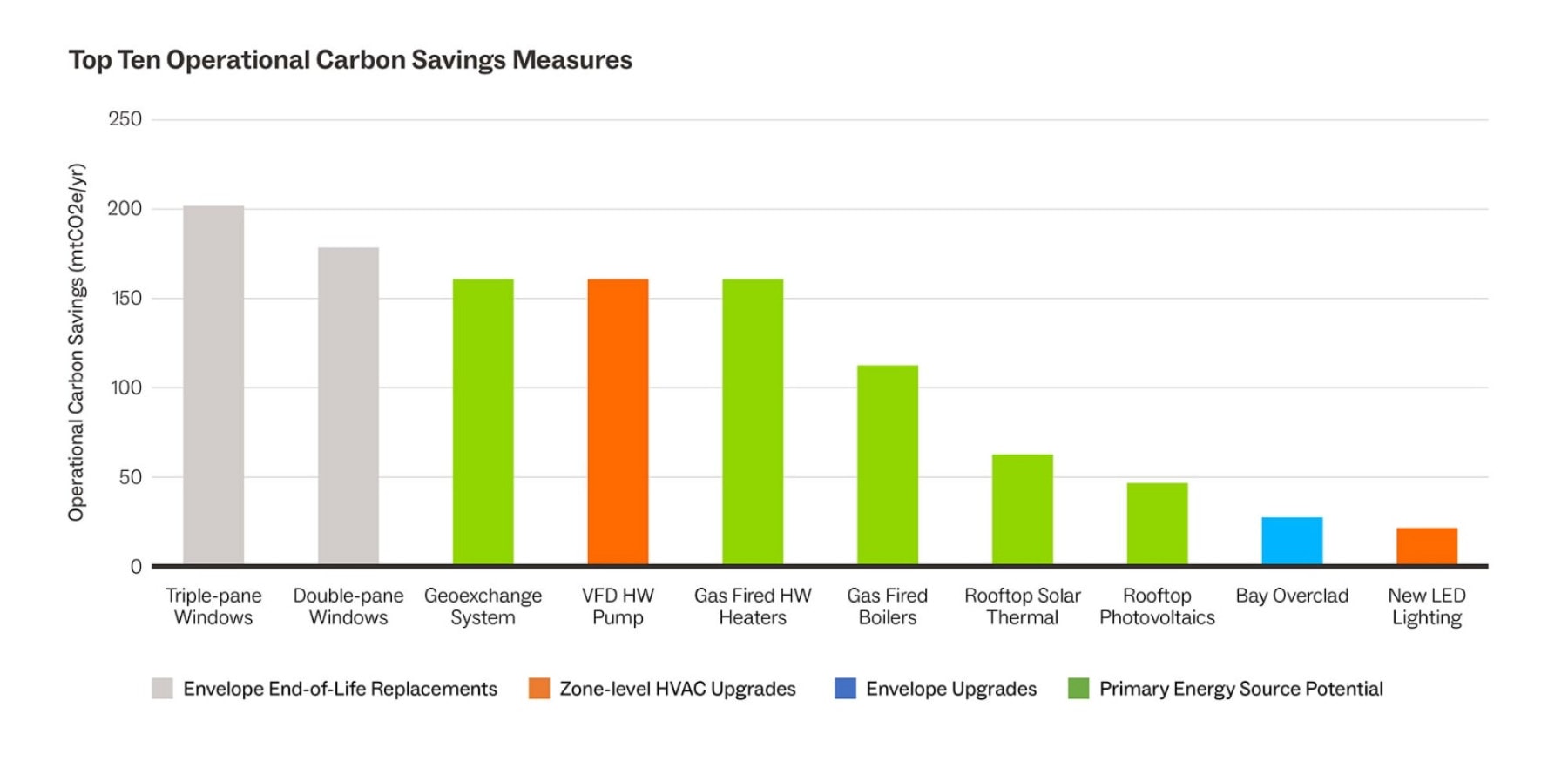
Case Study Analytical Results
The initial round of analytical modeling identified the two window upgrade options as the most significant measures for operational carbon savings, as shown in Figure 2. Of all the modeled envelope insulation configurations, the most effective option was an overclad intervention at the bay windows, while interior insulation offered only incremental benefits. Another key finding was the substantial diminishing rate of return on increasing the insulation R-values towards super-insulated levels.
The second round of modeling identified which measures would be most effective for reducing operational carbon in combination with each other and quantified the projected total carbon impact of each exterior envelope measure over time. As shown in Figure 3, the measures with the shortest carbon payback periods were envelope end-of-life replacement or refurbishment measures, while envelope upgrade options to add insulation at the typical existing wall condition had longer carbon payback periods. The exceptions were the envelope upgrade options using biogenic materials, some of which led to immediate total carbon reductions. For example, the interior insulation options using wood fiber insulation showed immediate carbon savings because the stored carbon of the wood fiber material was greater than the carbon emissions of the remaining materials included in the scope item.
While this study modeled an unusually in-depth analytical approach to selecting scope for a carbon-focused retrofit, some limitations to the process remain. First, the analysis was limited to the feasibility study phase, meaning that analysis and cost estimated were based on conceptual level information. The projected impacts to envelope performance, operational carbon, and embodied carbon of materials would continue to become more precise over the course of design before converting to actual emissions determined through construction, occupancy, and maintenance. For this reason, the study focused primarily on relative projected impacts rather than absolute projected impacts. While it is critical to establish a baseline existing performance to quantify savings of proposed scope items, it is not possible to create a comprehensive picture of existing energy and envelope conditions; even with extensive testing and investigations, some assumptions have to be made. Another analytical challenge was evaluating the individual and combined impacts of a large number of scope items simultaneously with the refinement and development of those same scope items. Due to embodied carbon data limitations around MEP components and time constraints, only envelope scope items were modeled for embodied carbon. A further iteration of this study would look at embodied carbon for all proposed scope items, particularly each energy conservation measure.
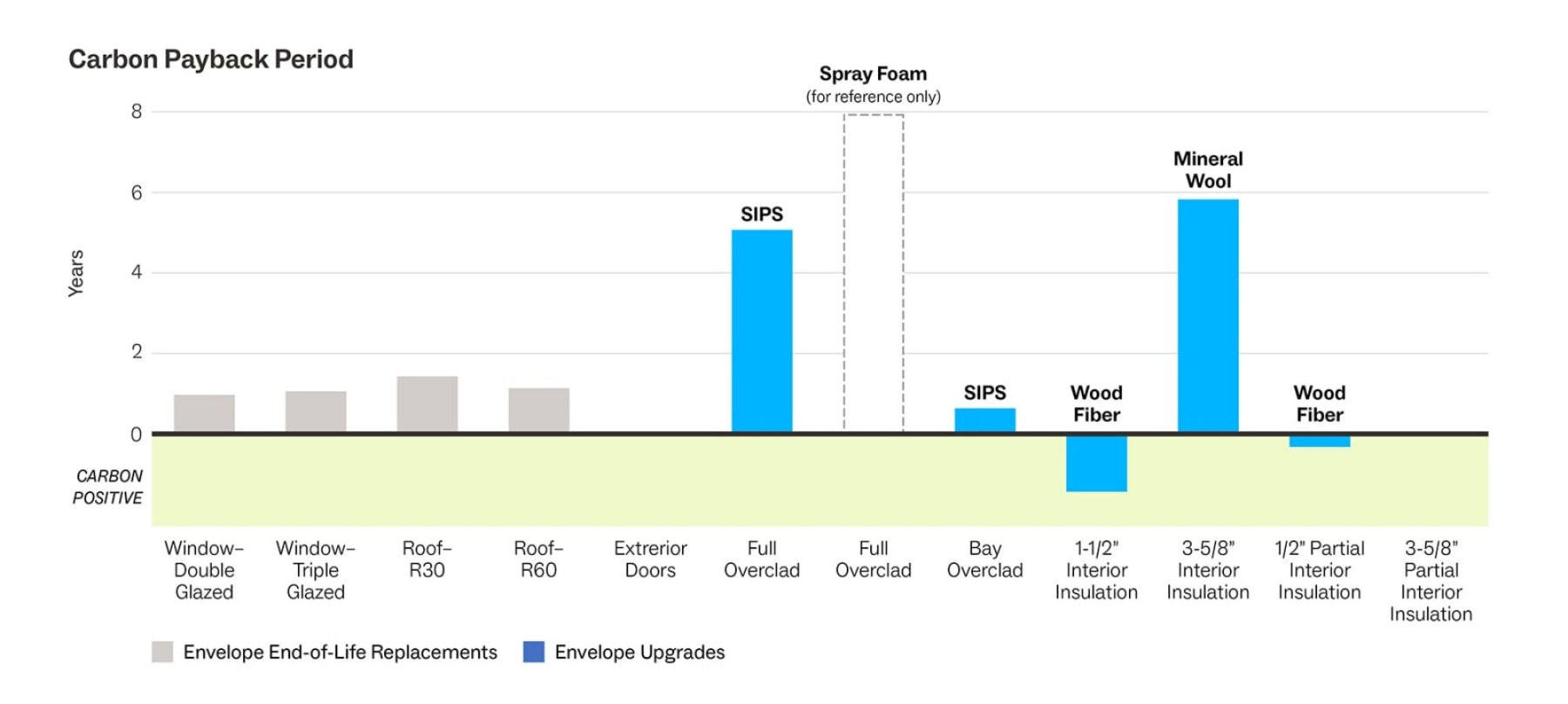
Recommendations
This study resulted in key findings on the impact of smart energy retrofit approaches that are broadly applicable.
- First, building envelope maintenance plays a significant role in reducing operational carbon. While regular maintenance is the best way to maintain air and water-tightness and minimize deterioration of components such as windows, deferred maintenance projects are crucial intervention moments–and afford opportunities to make carbon smart investments in a building. In the example of this case study, the single most effective measure for operational carbon savings was an envelope end-of-life component replacement. Evaluation of both embodied carbon of new components and resulting operational emissions reductions provide an opportunity to design for optimal carbon payback, ensuring true carbon reductions in the critical near-term.
- Second, super-insulating existing buildings does not always yield dramatic operational carbon savings. Overall building geometry and other existing physical constraints to the potential extents of new insulation can impact the carbon savings of adding insulation. For this reason, it is advisable to model insulation options for both typical exterior assemblies and identifiable thermal bridge locations to determine the most effective reductions in operational carbon, accounting for the unique conditions of individual projects. In some cases, discrete interventions at thermal bridges could be as or more effective than increasing the depth of an interior insulation cavity.
- Third, beyond avoiding carbon-intensive materials, options to store biogenic carbon through material selection should be explored for any applicable scope items.
- Last, operational carbon reduction potential is limited at the building scale. Building retrofits must be designed for compatibility with green energy sources to maximize carbon savings.
As retrofit projects are a vital piece of reducing global carbon emissions of the building sector, there is a clear need for a standardized approach to evaluating their total carbon impact. While practitioners are increasingly considering both embodied and operational carbon in project work, there is currently no standardized approach in the AEC industry to evaluate the two variables together. This study suggests two possible approaches that can be applied to either entire projects or individual scope items. One strategy is to minimize the carbon payback period. Another approach is to maximize the net carbon savings over a set period or before a particular target date in alignment with climate planning and regulation, such as the year 2030. As we approach critical milestones, the optimal payback timeframe will continue to evolve. This study underscores the need to shift away from a singular focus on annual operational impact relative to a performance baseline and towards total carbon impact as a function of time. Ultimately, the smart energy retrofit is one that considers the time value of carbon by tracking both embodied and operational reductions and minimizing total carbon footprint within the critical timeframe established to achieve global climate goals.
This piece was written in collaboration with Lori Ferriss, AIA, PE.